Nuclear Medicine
a brief introduction
Nuclear Medicine (NM) is based on the use of radioactivity for healthcare applications for diagnostic and therapy purposes. It makes use of biomolecules that are biomolecules that are attached to medical isotopes (radiopharmaceuticals ) to visualise and quantify numerous metabolic, molecular, and signalling pathways. Clinical applications of nuclear medicine include, for example, oncology, cardiology, neurodegenerative disease, and inflammation.
Nuclear medicine differs from other imaging procedures in that it determines the presence of disease based on the function of the organ, tissue or bone rather than based on structural appearance.
Nuclear Medicine may sound like a recent technology, but the science is far from new : it has been a vital imaging and therapeutic technique for almost a century! Today, it is more precise and sophisticated than ever thanks to a range of modern technologies that have evolved significantly, such as medical physics, radiochemistry (chemistry that deals with radioactive substances) and computer science.
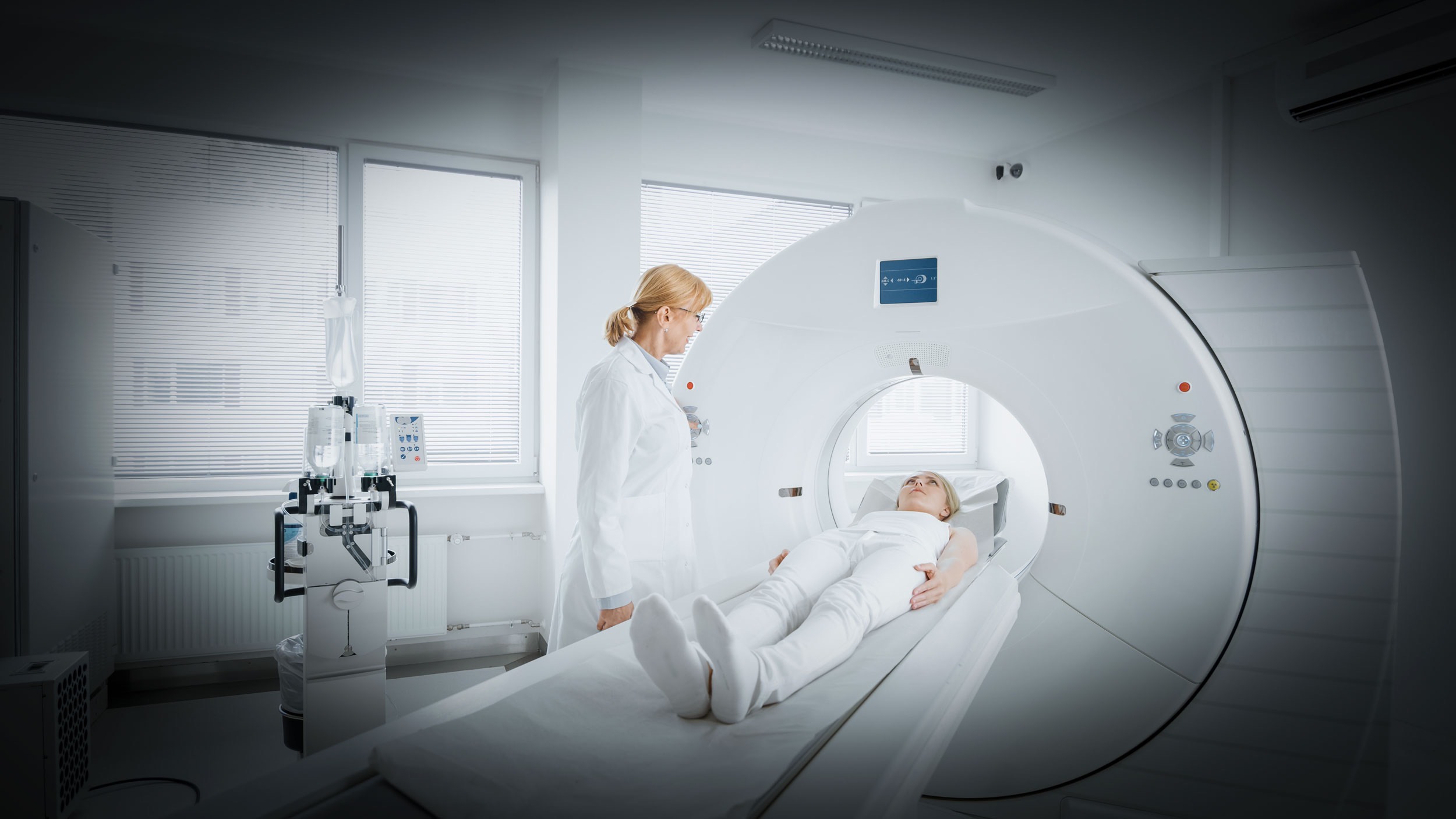
Nuclear Medicine Imaging
The medical isotopes as described above, send out radioactive signals. These signals can be detected by imaging techniques. In this way, Nuclear Medicine Imaging captures physiological or pathological pathways directly related to the disease status of the patient.
There are two types of nuclear medicine diagnostic techniques, Single Photon Emission Computed Tomography (SPECT) and Positron Emission Tomography (PET). The differences between the two techniques rely on the way the physical properties of the radiopharmaceutical are used.
Nuclear medicine differs from other imaging procedures in that it determines the presence of disease based on the function of the organ, tissue or bone rather than based on structural appearance. The advantage of assessing the function of an organ is that it helps physicians make a diagnosis and plan treatments for the part of the body being evaluated.
Today it’s possible to combine Nuclear Medicine Imaging with other imaging modalities like CT (SPECT/CT or PET/CT) or MR (PET/MR) for added diagnostic benefits. Similar terms might be used interchangeably: radiodiagnostics, molecular imaging, targeted radioligand therapies, and theranostics.
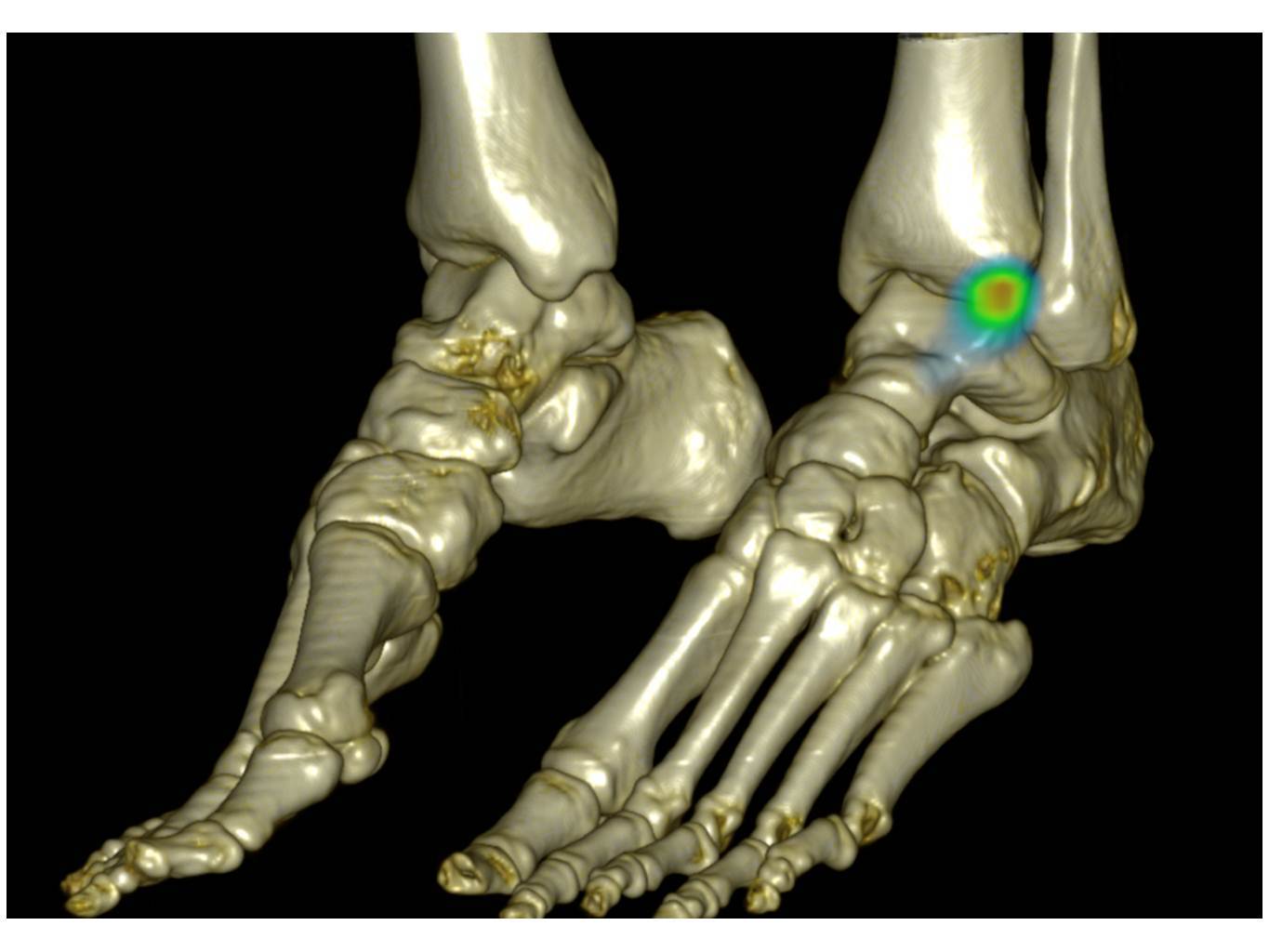
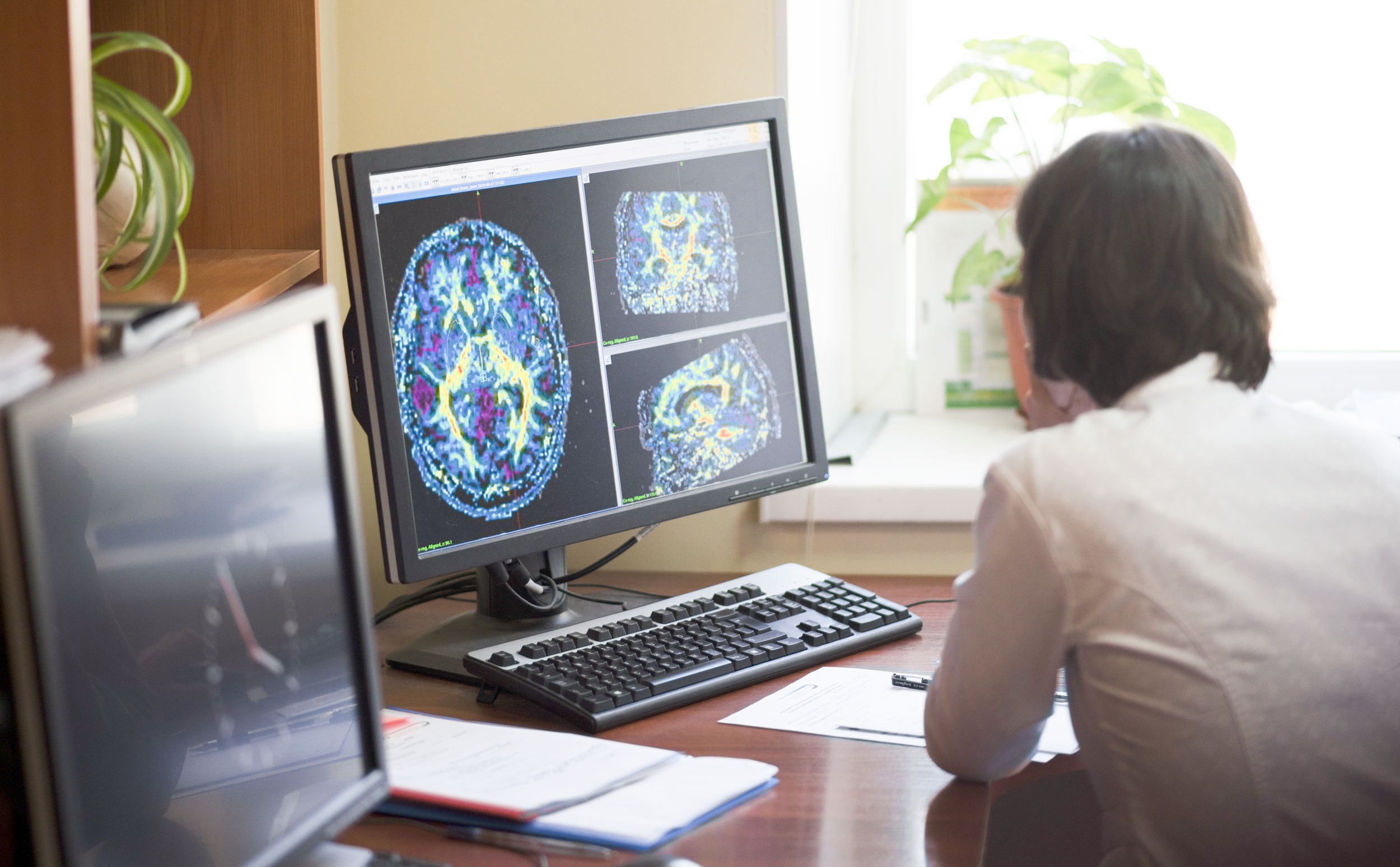
Nuclear Medicine Therapy
Nuclear Medicine Therapy enables a unique way of personalised treatment to cancer patients with a growing number of indications.
Theranostics: a unique feature of nuclear medicine!
The use of nuclear medicine provides a unique feature: theranostics, a combination of therapy and diagnostics. Theranostics is a newly invented word describing an emerging field within nuclear medicine.
Around the world, cancer affects millions of people. Unfortunately, there are many types of cancer with each type requiring a different treatment. An important challenge in cancer treatment is selecting the right drug or therapy approach, for that specific cancer and that specific patient. And that can kill the cancer cells in a targeted way, or in other words, to avoid harming the healthy cells in the body. Another challenge the physician has is to monitor the efficacy of the treatment: does the drug do the job as it is supposed to do and if not, adjust the treatment if possible? Theranostics helps physicians deal with these challenges.
This new technique combines therapy and diagnostics. The method uses one radioactive drug to detect (diagnose) the cancer and another radioactive drug to treat it (therapy). The drugs are designed to target specific molecules or pathways that are involved in the cancer’s growth and survival: these molecules and pathways identify the cancer cells for the drugs to be found. Due to their radioactivity, the drugs can be detected by special cameras. When these drugs find the tumours, the cameras show where the cancer is and how much of it is left.
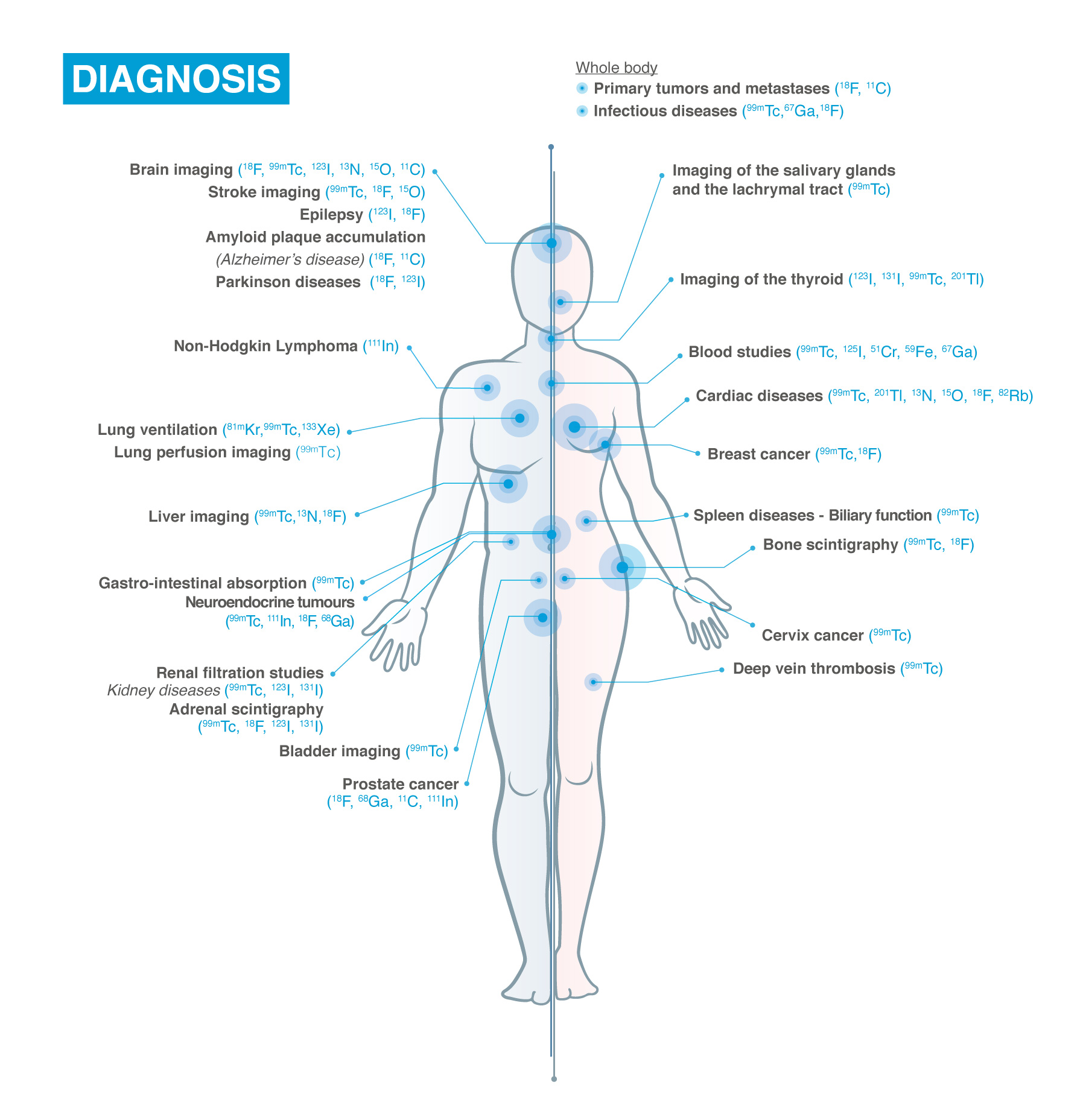
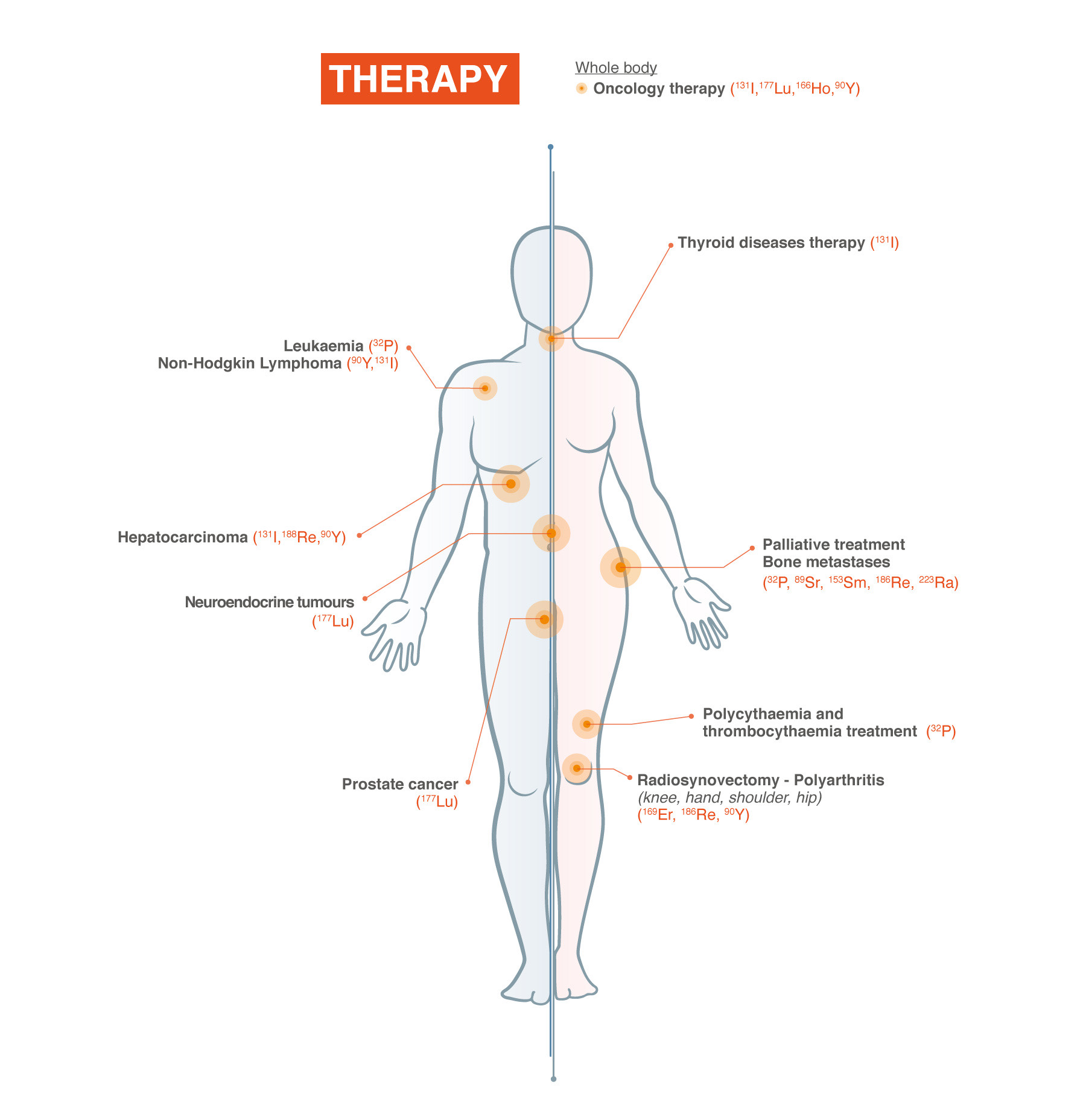
Some advantages of theranostics
over conventional methods of cancer treatment
- It can help doctors choose the best drug for each patient based on their tumour characteristics;
- It can help doctors monitor the response to the treatment and adjust the dose or switch to another drug if needed;
- It can help reduce the side effects of the treatment by minimising the exposure of healthy cells to radiation.
Theranostics is still a developing field that requires more research and clinical trials. However, it has shown promising results in treating some cancers, such as prostate, thyroid, and neuroendocrine tumours. Theranostics could potentially revolutionise the way we diagnose and treat cancer in the future. Not only is this technique used in oncology, but also other medical fields such as neurology and cardiology.
Similar terms might be used interchangeably: radiotherapeutics, radioligand therapies, and targeted radionuclide therapies.
Additional information for Patients
The General practitioner or a specialist physician will prescribe such an examination. A specialist physician could be a cardiologist, an endocrinologist, a urologist, etc. If hospitalised in a specific department, the specialist physician will prescribe such an examination when needed.
First, a radiopharmaceutical is administered to the patients (usually intravenously but sometimes orally), depending on which type of scan is being performed, the imaging will be done either immediately, a few hours later, or even several days after it’s administrated. Then, during the examination, the radiation emitted will be detected using a camera that works with a computer to produce images and measurements of organs and tissues.
Imaging time varies, generally ranging from 20 to 45 minutes.
After the procedure, a physician with specialized training in nuclear medicine checks the quality of the images to ensure that an optimal diagnostic study has been performed.
Nuclear Medicine exams are used in Oncology, Neurology, Cardiology and with the help of such exams scientists and healthcare providers are:
- gaining a better understanding of the pathways of disease
- quickly assessing new drugs
- improving the selection of therapy
- monitoring patient response to treatment
- finding new ways to identify individuals at risk for disease.
Most diagnostic investigations in nuclear medicine expose the patient to a small dose of radiation. The radiation dose is similar or even less compared to an X-ray (CT)
Nuclear medicine procedures are safe. Moreover, these procedures are painless, with extremely low incidence of side effects. The benefits definitely outweigh the risks.
There are no general rules for preparing for the nuclear medicine exam since each type of test has its requirements.
For example, one test may require you to not eat or drink – except for water – from six hours before the test until the test is complete. Another test may have no restrictions at all. The medical team from the institution where your exam will be performed will walk you through the journey and potential cautions before the exam.
Generally, you can resume your usual activities and normal diet immediately after the examination. The technologist will advise you of any special requirements or recommendations based on the type of procedure you had.
The website whatisnuclearmedicine.com provides an illustrated introduction to nuclear medicine, aiming to educate the general public about the role of nuclear medicine in both diagnostics and therapeutics.
The site contains detailed information on how nuclear medicine works, its applications in diagnosing and treating diseases, and the significance of radiopharmaceuticals in this medical discipline.
Additionally, it provides resources such as animations, posters and guides in multiple languages, for the patients, and for free.
This platform is dedicated to providing accessible and informative content on nuclear medicine for a general audience.
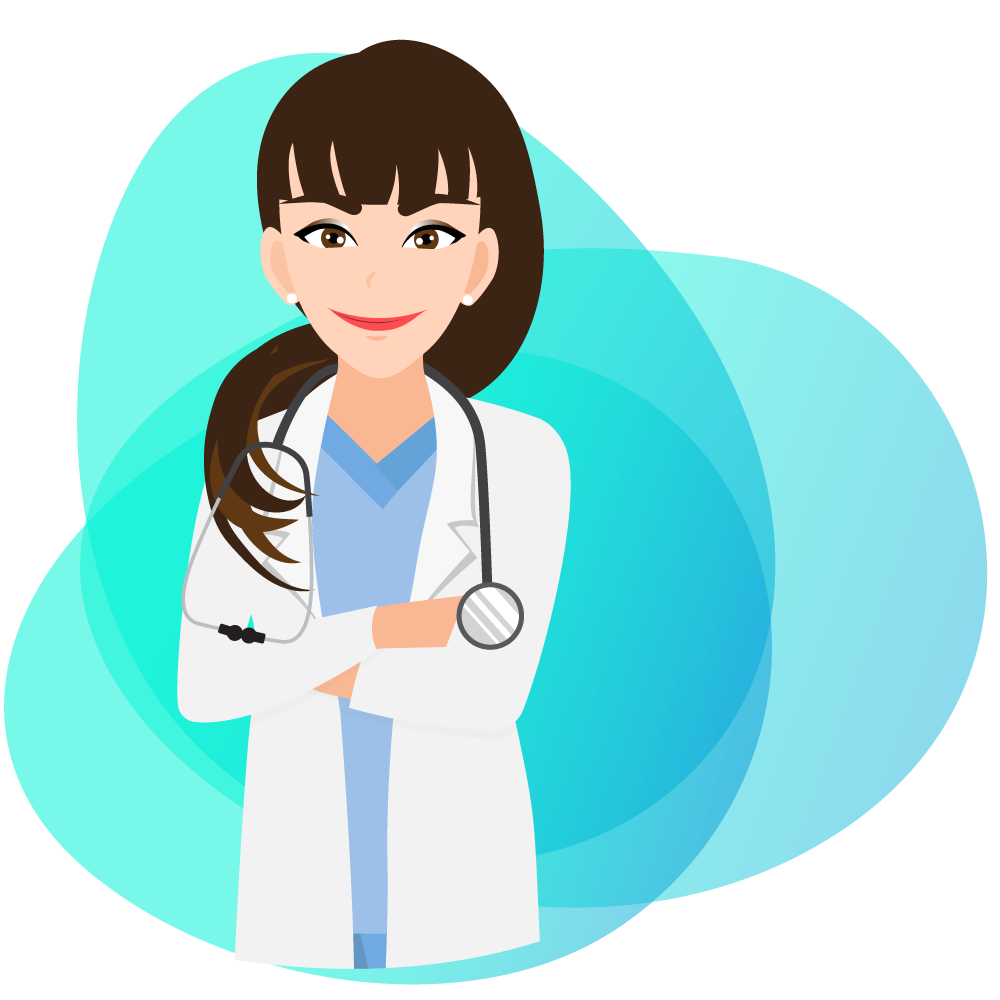
The content of this page has been crafted by the Nuclear Medicine Europe Communications Working Group